In view of the total solar eclipse of 2017 Aug 21 through the United States, this is a reminder of the role of solar eclipses in the developments of astronomy and astrophysics.
It is taken from a chapter of my book Glorious Eclipses, presented elsewhere in this blog.
Summary
Eclipses of the Sun and Moon have never ceased to provide us with a host of lessons about the nature of the universe around us. The first of these lessons concerned the celestial bodies directly involved in eclipses: namely the Earth, Moon, and Sun. Indeed, back in antiquity, the proof that the Earth was round, and the first measurements of the respective sizes and distances of the Moon and Sun were deduced from the observation of eclipses. In the 19th century, it was the normally invisible atmosphere of the Sun that was revealed thanks to eclipses. Far from being the perfectly round, and sharply defined ball of hot gas that it appears to the eye – appropriately protected by suitable filters, of course – the Sun is found to be a sprawling giant, overflowing with energy, plasma, and particles, that extends its influence throughout the whole Solar System. Eclipses also provoked the discovery of helium, the second most abundant element in the Sun, and in the universe as a whole. In a more surprising manner, in the 20th century, Einstein’s General Relativity, a fundamental theory about space, was tested experimentally for the first time, thanks to an eclipse. It is on this new vision of the universe, which explains gravitation in terms of the ‘curvature of space-time’, that all our current knowledge of the origin, the structure, and the evolution of the universe, depends, by way of the fascinating concepts of an expanding universe, the Big Bang, and black holes.
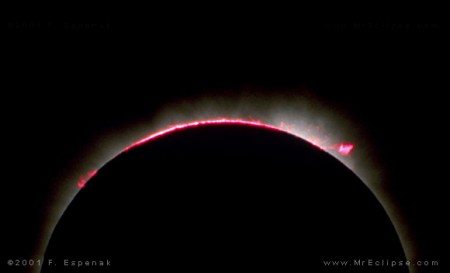
The Earth is round
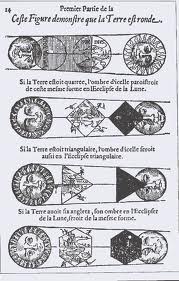
The first demonstration of an astrophysical nature resulting from eclipses is the one given by Aristotle concerning the fact that the Earth is round. The astronomical views of this Greek philosopher are well-known to us, thanks to his two works, known to us as Meteorology and On the Heavens, dating from the 4th century BC. Like other thinkers of his day, Aristotle believed that all heavenly bodies were spherical, because to him heavenly bodies were a reflection of divine perfection, and the sphere is the most outstandingly perfect geometrical figure. But this argument was not a physical demonstration, because, naturally, Aristotle did not have any experimental means of confirming the spherical nature of the planets and stars.
As far as the Moon was concerned, the philosopher adopted an explanation attributed to the Pythagoreans, namely that the observed appearance of the Moon throughout its various phases corresponded to a spherical body, half of which is illuminated by the Sun. As for the spherical nature of the Earth, the proof given by Aristotle is quite original: he notes that an eclipse of the Moon is caused by the shadow of the Earth, and that the circular shape to the edge of the shadow seen on the Moon’s surface implies that our world is spherical
Sizes and distances of the Moon and Sun
The golden age of Greek astronomy flourished at Alexandria. Since its foundation under the reign of Ptolemy Soter (3rd century BC), the Alexandrian school brought together brilliant mathematicians and geometers, such as Euclid, Archimedes, and Apollonius. Similarly, the greatest ancient astronomers Aristachus of Samos, Eratosthenes, and Hipparchus, as well as Ptolemy (2nd century BC), all worked there.
Aristarchus (310-230 BC) is nowadays known for having been the first to voice the heliocentric theory, i.e., that it is the Sun that reigns at the centre of the world system, not the Earth as was believed at the time. His statement does not appear in any known work, but it was reported by Archimedes and by Plutarch. The only work of Aristarchus that has come down to us relates to the sizes and distances of the Sun and the Moon.
The Alexandrian astronomer completely reopened this question, which had been discussed since the 4th century BC. The Pythagoreans had positioned the heights of the celestial bodies according to musical intervals. Eudoxus, the brilliant disciple of Plato, had estimated the diameter of the Sun as nine times that of the Moon. As for Aristarchus, he devised an ingenious geometrical method of calculating the distance ratios of the Sun and Moon.
He found that the Sun lay at a distance between 18 and 20 times that of the Moon. (In fact, it is 400 times as far.) By an argument based on the observation of eclipses, he determined the diameter of the Moon as one third of that of the Earth, which is very close to the actual value. He also announced that the diameter of the Sun is seven times that of the Earth. Even though Aristarchus considerably underestimated the size of the Sun, because it is actually 109 times as large as the Earth, he had grasped the essential fact that the daytime star was much larger than the Earth. It was precisely this result that led him to the heliocentric hypothesis. He did, in fact, argue that under these circumstances, it was logical to believe that the Earth and the other celestial bodies revolved around the Sun, rather than the reverse. Aristachus was before his time. The world had to wait until 1543 and the work by Copernicus, before the heliocentric theory was again put forward, this time with success.
A century after Aristachus, and again at Alexandria, Hipparchus developed a complete theory of the Moon. He defined the lengths of the synodic month (or lunation, the period in which the Moon returns to the same position relative to the Sun); the draconitic month (the period for the Moon to return to the same position relative to the nodes of its orbit); and the anomalistic month (the period for the Moon to return to perigee or apogee). The immense improvements that Hipparchus brought to theories of the apparent motion of the Moon and Sun enabled him to have far more success than his predecessors in dealing with the problem of predicting eclipses, which had always been of immense interest.
Hipparchus considerably extended Aristarchus’ method: by observing the angular diameter of the shadow of the Earth at the Moon’s distance during a lunar eclipse, and comparing it with the known apparent diameters of the Sun and Moon (about half a degree), he obtained the ratio of the Earth-Moon and Earth-Sun distances, giving one when the other is known. Pappus, another famous astronomer of the Alexandrian school, recounts that Hipparchus made the following observation of: “An eclipse of the Sun, which in the area of the Hellespont was precisely an exact eclipse of the whole Sun; such that none of it was visible, but at Alexandria, in Egypt, about 4/5 of its diameter were hidden. By means of the foregoing arguments, [Hipparchus] showed that, measured in units where the radius of the Earth has the value of 1, the smallest distance to the Moon is 71, and the larger 83. Whence the average of 77.“
The total solar eclipse mentioned is that of 20 November 129 BC. The actual value of the Earth-Moon distance is 60,4 terrestrial radii.
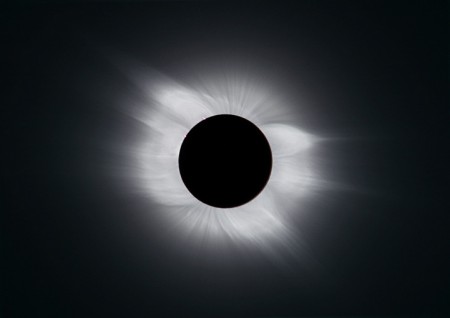
The empire of the Sun
The name ‘photosphere’ is given to the layer of gas that provides most of the light from the Sun. Its limit, seen in profile, forms the apparent edge of the disk. During a total solar eclipse, however, the Sun’s invisible empire is revealed: its two outer atmospheres, the chromosphere and the corona, appear as a irregular halo.
The chromosphere extends from the photosphere up to a height of about 15 000 km. Its name arises from the fact that the emission lines in its spectrum were originally detected in different ‘colours’ of the visible spectrum, which – as we may recall – may be dispersed to give the colours of the rainbow, from red to indigo. The chromospheric lines are red, green, blue, and violet from hydrogen, and yellow from helium. The chromosphere is the region in which eruptions and prominences occur.
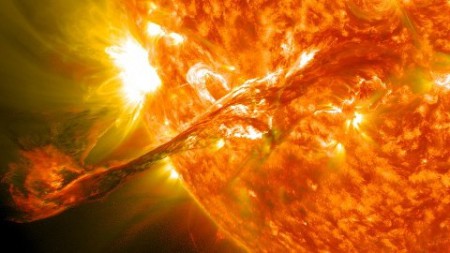
As regards the corona, it is the outer, highly tenuous, region of the Sun’s atmosphere. It may be sub-divided into two layers, the inner corona between 15 000 km and 200 000 km above the photosphere, and the outer corona beyond that. It consists of negatively charged electrons, and positively charged atoms known as ions. Under normal conditions, atoms are electrically neutral, but when they are raised to high temperatures, they lose their electrical neutrality together with their electrons, and are ionized. This is what happens in the solar corona. This flux of charged particles is ejected into interplanetary space by the Sun, and forms the solar wind. When it reaches the vicinity of the Earth, the solar wind is still blowing at about 400 km/s. Its particles are channelled by the Earth’s magnetic field and may cause perturbations – the notorious geomagnetic ‘storms’ – and polar aurorae.
The corona consists of plumes, filaments and streamers of pearly white light. Although it is one of the most beautiful and spectacular aspects of the Sun, it is so pale that it is invisible under normal conditions. In fact, although the corona is extremely hot – two million degrees – it is also very tenuous. In visible light it radiates about one millionth of the light from the main surface, the photosphere. It is, as a result, completely drowned by the latter’s brilliance, so that none of it whatsoever may be seen.
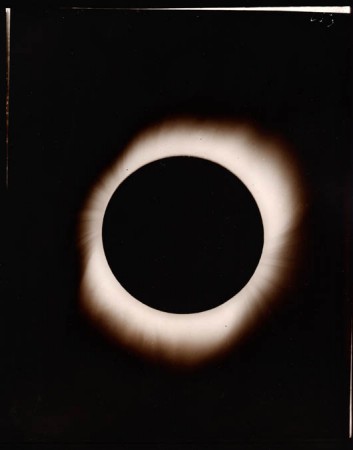
A total eclipse of the Sun is an astronomical event that offers the possibility of seeing the corona in all its splendour. At the precise moment when the disk of the Sun vanishes, this incandescent halo suddenly appears, like a vast fan of light, with a diameter about twice that of the solar disk. Some of its jets may be followed with the naked eye out to around six solar radii. This is the reason why total eclipses are so sought-after by professional solar physicists, who do not hesitate to organise expeditions to the other side of the world to take advantage of those few moments of observation.
The true nature of the solar atmosphere was not understood until the second half of the 19th century. Before then, the existence of a luminous halo visible only at eclipses was mentioned by Plutarch in the year 98, and discussed by Kepler in his Epitome on Copernican astronomy in 1621. A coloured halo was also described by Halley during the eclipse of 1715, in the form of a narrow red band. But astronomers all made the error of thinking that it was either an optical illusion, scattering caused by the Earth’s atmosphere, or evidence of a lunar atmosphere.
The idea of an atmosphere of the Sun itself took shape in the 18th century, with Dortous de Mairan and Cassini. Their observations were not of eclipses but concerned the zodiacal light. The zodiacal light is a faint, night-time glow, which may be detected on a clear, Moonless night. Centred on the plane of the ecliptic – the Sun’s apparent path across the celestial sphere, during which it crosses the constellations of the Zodiac, whence the term – the zodiacal light is nowadays understood to be caused by the scattering of sunlight by the cloud of interplanetary dust that surrounds the Sun. In this way some of the regions of the Sun’s empire that are ordinarily hidden come into view.
The first exhaustive study devoted to observation of the solar halo was carried out by Francois Arago, at the total eclipse of 1842. Clearly describing the presence of the halo, he suggests that it should be called the ‘lunar corona’, thus perpetuating the error that had already been committed by his distinguished predecessors.
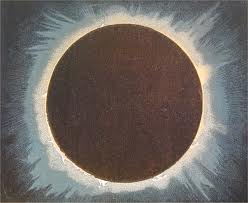
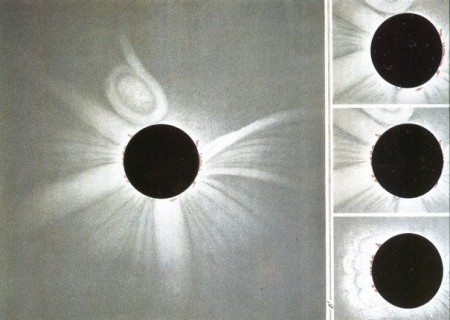
It was not until the eclipse of 28 July 1851 that the halo, photographed for the first time by Berkowski, was definitely shown to be physically associated with the Sun. The situation was confirmed in 1860, during an eclipse observed from Spain. Warren de la Rue and Angelo Secchi obtained daguerrotypes of the solar halo from two sites on the path of totality, but 500 km apart. The structures seen during the eclipse were similar on both photographs. There was, therefore, no parallax effect. Parallax is the change in perspective when an object is seen from two different positions. If you hold your finger up in front of your nose and alternately close the right and left eye, your finger moves relative to the background. If you increase the distance to your finger, by extending your arm, and try it again, the finger still moves, but less distinctly: the farther the finger is away, the smaller the movement. The experiment by de la Rue and Secchi observed solar prominences with eyes 500 km apart. The absence of parallax proved that these structures did not belong to the Moon, which is too close, but to the Sun, which was sufficiently far away for the change in perspective to be imperceptible.
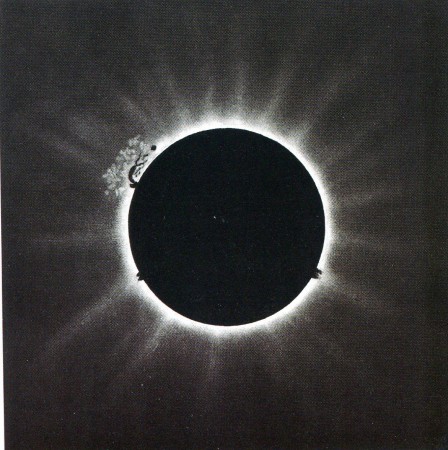
During the total solar eclipse of 18 August 1868, the spectrum of the prominences and chromosphere was obtained for the first time by Jules Janssen, the future founder of Meudon Observatory, and by Norman Lockyer. The spectrum proved to consist of bright lines, demonstrating that the source of the emission consisted of gas. The most important lines present in the spectrum of the chromosphere were those of hydrogen, two lines belonging to calcium, and a yellow line of a hitherto unknown element. Believing that it was an element unique to the Sun, it was called ‘helium’ (from the Greek helios, Sun). Helium was discovered on Earth in 1895. Air contains 0.0005% by volume. It is a ‘noble gas’, very light, and therefore used to inflate balloons. Although it is rare on Earth, helium is, in fact, the second element in the periodic table and is, after hydrogen, the most abundant element in stars and the universe as a whole: it amounts to 25 % of the total mass.
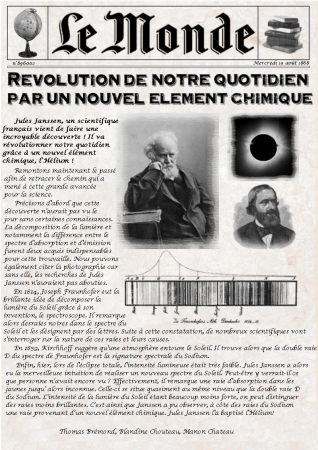
During the course of a solar eclipse, at the exact moment when the western edge of the- Moon hides the edge of the photosphere, the chromosphere appears as an irregular fringe, partly caused by its structure, and partly because of the lunar relief. The latter is responsible for a beautiful and spectacular phenomenon, that of Baily’s Beads. The name goes back to 15 May 1836, when the British astronomer Francis Baily observed an annular eclipse of the Sun, and noticed irregularities on the lunar limb. He described them as like a necklace of beads of light, and gave the true explanation: sunlight is blocked by lunar mountains, but passes through the intervening valleys. Generalizing, the complete ring of Baily’s Beads appears whenever the diameter of the Sun lies between the minimum diameter of the Moon (corresponding to the bottom of the valleys) and its maximum diameter (corresponding to the mountain peaks). Baily’s Beads were made a particularly great impression on the crowds that gathered at Saint-Germain, near Paris, for the annular eclipse of 17 April 1912.
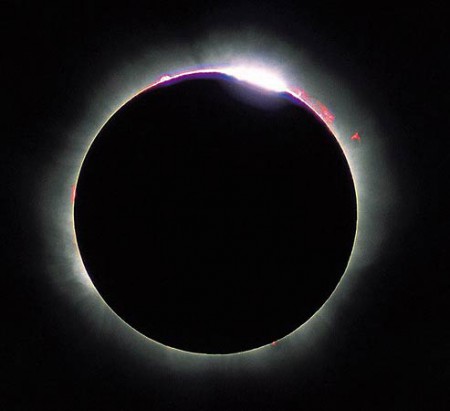
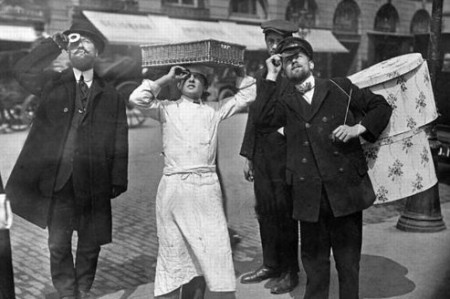
During an eclipse, the Moon, which continues to move, takes a few seconds to cover the chromos-pheric fringe at the eastern limb of the Sun before revealing that on the western limb, at the end of totality. Astronomers thus have about ten seconds at the beginning and ten seconds at the end of an eclipse to photograph the upper chromosphere. To capture the lower chromosphere, which lies between 2000 and 3000 km above the photosphere, the time available is even shorter. It requires a flash spectrum, using a slitless spectrograph – the chromosphere being sufficiently narrow to act as a slit itself.
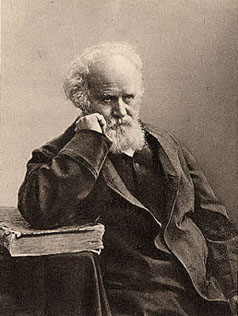
As for the corona, spectroscopic analysis also produces surprises. During the eclipse of 1869, Harkness and C.A. Young discovered a spectral line in the green region. They attributed it to an unknown element, called ‘coronium’. But observations made by Jules Janssen in Hindustan in 1871 and from the Pacific in 1883, identified this line as that of a well-known element: neutral hydrogen. Throughout his life, Janssen showed a remarkable tenacity in observing eclipses. In 1870, when Paris was besieged by the Germans, he escaped in a balloon to observe the eclipse of 22 December. When he arrived safe and sound at Oran, in Algeria, where he went ashore to observe the eclipse, clouds completely covered the sky!
The artificial eclipse: Lyot’s coronograph
Because of the extreme brevity of total solar eclipses (just a few minutes), scientists thoughts soon turned to observing the corona outside eclipses. But the difficulty is considerable. Not only is the brightness of the corona in normal light just one millionth of that of the photosphere, but the sky in the vicinity of the Sun’s disk is itself ten to one hundred times as bright as the corona, because of scattering of sunlight in the Earth’s atmosphere.
In 1931, the French astronomer Bernard Lyot invented an instrument that enabled one to create artificial eclipses at will, to observe the corona in daylight and continuously. To do this, it is not sufficient to use just a black disk that exactly masks the photosphere. His coronograph also suppresses the stray light scattered by the optical elements in the instrument. It enabled Lyot to carry out daily visual, photographic, and spectroscopic observations of the inner corona.
In 1933, Lyot perfected his instrument by adding a monochromatic filter that selected the appropriate radiation for different layers of the Sun. He was thus able to obtain photographs of the Sun at three important wavelengths, including the Ha (H-alpha) line of hydrogen. With this equipment, it is possible to make a film of prominences. From 1935, Lyot obtained some magnificent films, such as one called Flames on the Sun, showing the jerky movement of prominences and the fireworks of eruptions.
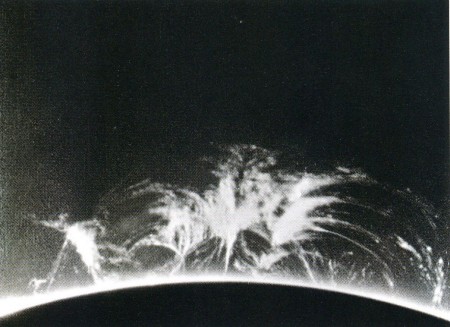
The author Claude Roy described the discovery in these words: “Thanks to the coronograph that he [Professor Lyot] has invented, I have seen, erupting from the Sun’s corona, and from its outer surface, the great sheaves of solar eruptions, those feathery plumes of gas in the act of fusion, whose vigour is measured […] in hundreds of thousands of kilometres.“
The jewels in the crown
Many observatories around the world now have coronographs and spectroheliographs. Some sites, such as the Pic du Midi in France, Pico de Teide in the Canary Islands, and Sacramento Peak in New-Mexico, have even specialized in the study and surveillance of the Sun. Nevertheless, whatever the quality of the coronographs, only the inner regions of the corona are detectable outside eclipses. A total eclipse, which is the best of all coronographs, also allows observation of the outer corona, especially if one uses instruments mounted on aircraft that fly at high altitudes, where the rarified atmosphere is transparent and scatters very little of the sunlight. This is why, for 30 June 1973, which was to be a very long eclipse – 7 minutes 4 seconds – a French team led by Pierre Lena and Serge Koutchmy suggested that a suitable flying observatory would be – Concorde 001. The aircraft took off from the Canaries, caught up with the eclipse as it sped across Africa, and, flying at 2200 km/h, remained within the Moon’s shadow cone for 74 minutes, while ten scientists studied the corona through portholes that had been specially polished for the occasion. Despite the duration and the altitude record – 17 000 m – of the observations, atmospheric turbulence, partly caused by the aircraft’s supersonic Shockwave, did limit the scientific value of this spectacular mission.
Total solar eclipse 1973-06-30 viewed from Concorde plane; Concorde hunted by totality over Africa (Mauritania)
In general, before the advent of space missions, like Skylab in the 1970s and, above all, Soho from 1995 onward, astrophyicists wanting to record spectra and images of the solar corona were forced to travel, with all their scientific equipment and baggage, to places where eclipses were to take place. On 11 July 1991, however, the Sun and Moon offered them a fine present by visiting their own backyard, namely one of the most important observatories in the world, which is located on the top of Mauna Kea, on the island of Hawaii. This international site, perched at an altitude of 4200 m, is equipped with extremely powerful telescopes, whose sole fault is that they were not designed for observing the Sun! As a result, the scientists had to adapt instruments designed to observe galaxies one hundred million times as faint as the faintest stars visible to the naked eye, so that they could observe our star as it was eclipsed. A whole host of precautions had to be taken to protect the telescopes. The most powerful of them, the Canada-France-Hawaii Telescope, has a mirror 3.6 m in diameter. The slightest ray of light from the photosphere that struck this mirror would turn it into a solar furnace. So, a perfectly opaque mask, with just two minute pinholes, which enabled the Sun to be located and followed without danger, was placed in front of the mirror until just a few seconds before totality. At the exact moment when the last ray of light from the Sun disappeared, the mask was removed. For the four minutes that the eclipse lasted in Hawaii, the CFH Telescope was thus able to provide its full optical power. Never had such a powerful instrument been used to study the Sun. This time, the gamble paid off for the international team using the telescope, led by Serge Koutchmy from the Institut d’Astrophysique in Paris: the mirror’s exceptional optics enabled them to photograph extremely fine structures, less than 300 km across, within the corona. This was a surprise to the physicists, who had never imagined that such small structures could survive in a medium that was so tenuous, at such a high temperature, and moving so rapidly. These discrete coronal threads, as Koutchmy has called them, have a very short lifetime of about one minute. During this memorable observation on 11 July 1991, Serge Koutchmy’s team were also able to capture a solar structure, 1500 km in diameter, that had been completely unknown until then, and which he subsequently called a ‘plasmoid’. It is a bubble of cold plasma, which originates at the surface of the Sun, and rises into the corona, before dissipating. A high-resolution film obtained with the CFH, centred on a moving plasmoid, shows the latter becoming distorted and breaking up. The movement of the plasmoid is not radial to the Sun, but followed the local magnetic field.
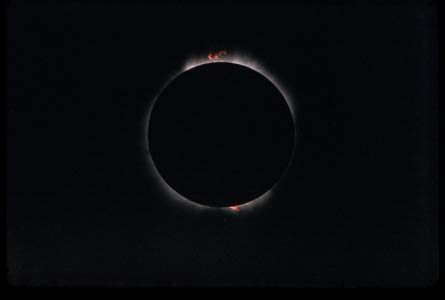
In a dome adjacent to the Franco-Canadian telescope, another team observed the eclipse with the University of Hawaii’s 2.2 m telescope. Here again, the researchers had the use of an extremely powerful telescope to study the Sun. They put it to good use during the four minutes of totality by making a high-resolution film of a giant solar prominence, itself located, before the start of the eclipse, by the coronograph at a neighbouring solar observatory on Mauna Loa. Without this valuable contribution, the astronomers would never have had the time, with their large telescope, to ‘tour the Sun’ to find an interesting feature – the field of view of a telescope 2.2 metres in diameter is actually very tiny. Once the eclipse was over, computer enhancement of the original images, designed to improve their resolution, was applied to the film. The astronomers used the lunar limb, which should be extremely sharp, as a reference. They were thus able to partially correct for atmospheric turbulence that distorted the images as they were being taken. This spectacular film led to a better understanding of the fine structure of solar prominences, which had never been observed under such conditions.
The shape of the corona varies from eclipse to eclipse, depending on the Sun’s magnetic activity. This follows an 11-year cycle, which is particularly well shown by the appearance, migration, and disappearance of dark sunspots on the photosphere. At solar maximum -corresponding to a maximum number of sunspots – the corona is regular, almost circular, in shape. At solar minimum, its shape is very irregular, and takes the form of extremely extensive equatorial streamers, which sometimes stretch out as far as twelve solar diameters, and which give the Sun a ‘winged’ appearance. The existence of the Sun’s overall magnetic field, which determines the shape of the corona was suggested in 1889 by Bigelow, and confirmed in 1891 by A. Schuster. As the latter said, the shape of the corona around minimum is ‘very similar to what one would find if the Sun were a magnet, discharging negative electricity around the poles’.
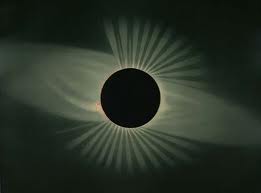
Since it has become possible to observe the corona thanks to telescopes in space, scientific interest in eclipses has diminished. Outside the Earth’s atmosphere, in fact, the sky background is completely dark, atmospheric scattering has disappeared, and the corona is distinctly seen. Spaceborne experiments, which have extended observations of the corona out to thirty solar radii, i.e., 20 million kilometres from the Sun’s limb, have confirmed Schuster’s intuition. They have also allowed us to define the physical properties of the transition zone between the Sun’s atmosphere and the interplanetary medium.
The European spaceprobe Soho, launched on 2 December 1995, has been working between the Sun and the Earth, at 1.5 million kilometres away from the latter, where there is a point of gravitational equilibrium between the two bodies. Equipped with 12 instruments designed to study the solar wind, the Sun’s atmosphere, and the body of the Sun itself, in different bands in the visible and ultraviolet regions of the spectrum, Soho has made numerous discoveries. These have . included the existence of broad currents within the Sun’s interior near the poles, and also allowed us to understand what had been the greatest mystery of solar physics until now: why are the temperatures found in the Sun’s outer atmosphere so high? While the temperature at the Sun’s surface is around 5000°C, it climbs to 2 million degrees above 10 000 km. Scientists have never been able to account for this extraordinary heating of the corona. A mechanical explanation was suggested in 1942 by Martin Schwarzschild, who had detected the extreme agitation of the solar surface at kilometre wavelengths: material was flowing upwards at velocities that reached hundreds of kilometres per second. The currents and the immense Shockwaves that they produced were believed to explain the heating, but only in part, because the amount of energy involved remained insufficient.
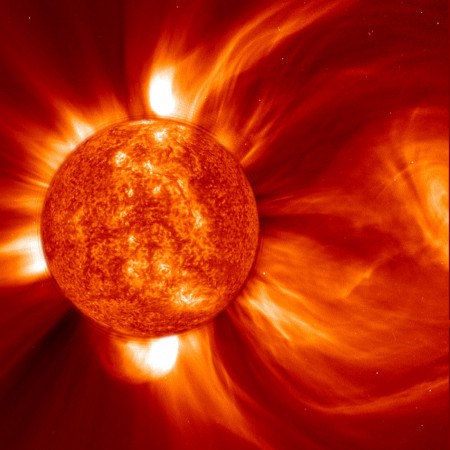
Soho revealed a new aspect of solar magnetism: the dynamo effect. This phenomenon explains the origin of the magnetic fields through the rotational and convective motions in the fluid, conductive interior. Its role in the Sun nowadays appears to be much greater than previously thought, because it may also explain the heating of the corona. A continually changing ‘magnetic carpet’ of lines of force, which creates magnetic currents incomparably greater than on Earth, is woven throughout the Sun and its atmosphere. Imagine an electrical network that is constructed by connecting powerful generators through good conductors: such a network would heat up. Similarly, the Sun’s magnetic network causes the strongest heating just where the loops are closed, that is, at altitude.
Eclipses and the curvature of space
Eclipses are a phenomenon that involves the Earth, the Moon and the Sun: three bodies that occupy a derisively small region of space when compared with the immensity of the Galaxy, never mind the universe as a whole, with its hundreds of billions of galaxies. Yet eclipses have allowed us to establish, on a true experimental basis, the fundamental theory about the nature of space, time, matter, and light: general relativity. This has literally changed our vision of the cosmos, notably by leading to the concept of a universe that is expanding from an initial Big Bang.
In 1905, Albert Einstein published three papers, each worthy of a Nobel Prize. One explained Brownian motion, another the photoelectric effect, and the third advanced the theory of Special Relativity. The success of these papers transformed an obscure employee in the Patent Office in Berne into one of the foremost among the small group of physicists. This phenomenal start was followed by other major advances, but what was by far the most influential of his work was the theory of General Relativity, which describes the distortion of space-time by a gravitational field. In 1911, when he was working at the University of Prague, Einstein made the first calculations, but he did not take any astronomical data into account. It was the German astronomer Erwin Findlay Freundlich who convinced him of the importance of observations that would test his theory of gravitation, notably the deviation of light rays near the Sun. According to General Relativity, the curvature of space near a massive body should cause rays of light passing nearby to curve inwards. A star seen at the edge of the Sun’s disk at the time of eclipse, would thus appear to be shifted through a certain distance relative to its normal position.
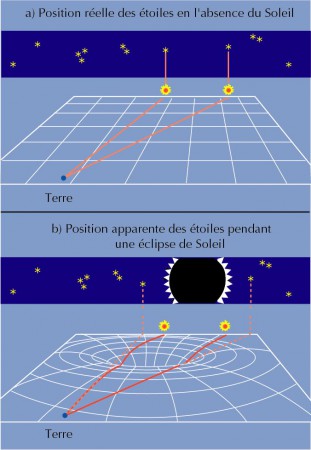
A useful image of curved space-time, and of its influence on the motion of light and matter, is that of an elastic sheet. Imagine a piece of space-time reduced to two dimensions, in the form of an elastic sheet. In the absence of any object on top of it, the sheet remains perfectly flat. If one places a ball on it, the sheet deforms, and creates a hollow around the ball. The heavier the ball, the deeper the hollow. If, in addition, the sheet is actually a network, the threads in the net allow us to visualize the paths that rays of light will follow. They form a perfectly rectangular array in the absence of gravitation, but deviate from a straight line in the presence of any mass, following the warped shape of the sheet. This deviation of the rays of light passing close to the Sun is one of the specific effects of General Relativity predicted by Einstein that may be verified experimentally. It should be revealed by a slight displacement in the position of stars when the Sun is close to their line of sight. Naturally, no one could expect to observe such a phenomenon except during a total eclipse of the Sun.
A German expedition to the Crimea was arranged for the 21 August 1914, the date of a solar eclipse. But that was not a judicious time to be a German on Russian territory. The team was imprisoned for a month until an exchange could be arranged, and no scientific photographs were taken. Some historians of science have noted that it was perhaps just as well: the calculation of the deflection of light that Einstein advanced in 1911 was in error by a factor of 2, and the Crimean observations would have contradicted his prediction. Knowing Einstein’s personality, however, we may well imagine that such a reverse would not have discouraged him.
However that may be, in the meantime Einstein had realized that his theory was inaccurate. He finally succeeded in formulating it in 1916, in particular calculating that the true value of the deviation of rays of light at the Sun’s limb would be 1.74 seconds of arc.
During the First World War, few scientific ideas were exchanged between the Germans and the Allies. When the British astronomer Arthur Eddington received Einstein’s paper on General Relativity ‘through the grapevine’, he immediately realised its importance.
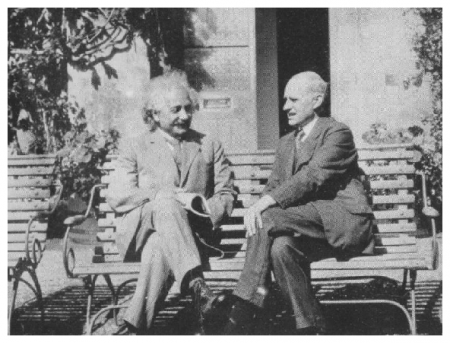
A total solar eclipse was due to occur on 29 May 1919, along a line passing through Brazil, the Atlantic, and West Africa. Eddington and his colleague Frank Dyson organised an expedition with the aim of detecting the effect predicted by Einstein’s theory. The moment when Eddington proposed the expedition corresponded to the peak of the war, when prospects for the Allies appeared at their darkest. A British test of a German theory would be a major proof of the internationalism of science. But Eddington was a Quaker, and thus a confirmed pacifist, and he gained his ends.
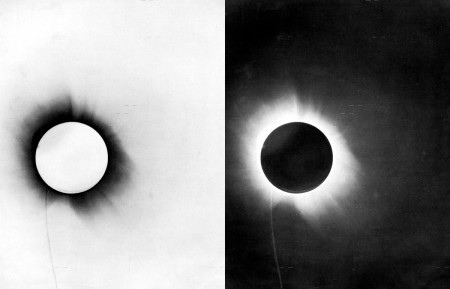
To better guard against the risk of bad weather, Eddington and Dyson chose two sites: one on the island of Principe, on the West Coast of Africa, which was where Eddington went, and the other at Sobral, in Brazil. At Principe, on the day of the eclipse, it started to rain heavily; the rain ceased at midday, but the Sun did not appear until after first contact. Nevertheless, Eddington took 16 photographs through scattered clouds. Six months later, he returned to the site to take the same field of view, in the absence of the Sun, i.e., at night, so that the apparent positions of the stars could be compared. The results confirmed Einstein’s theory, giving values of 1.98 + 0.30 arc-seconds and 1.61 + 0.30 arc-seconds. They were announced on 6 November 1919 at a memorable meeting of the Royal Astronomical Society. The audience fully realized that they were witnessing a turning point in the history of physics. The international press trumpeted relativity’s success, and Einstein became the symbol of scientific genius.
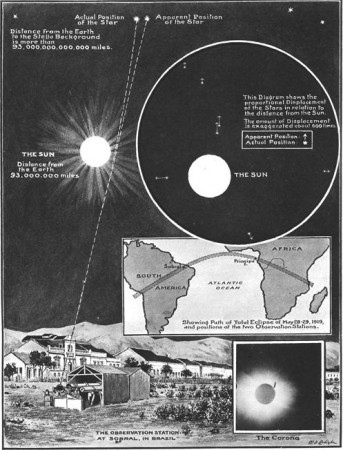
Einstein was not surprised. He later told the tale of how he was in the company of Max Planck, the German physicist who played a pioneer role in the theory of quantum mechanics. “He was one of the most intelligent people I have ever known; but during the 1919 eclipse, he stayed up all night to see if it would confirm the deviation of light by the Sun’s gravitational field. If he had really understood the way in which General Relativity explains the equivalence of inertial mass and gravitational mass, he would have gone to bed like me!“
Albert Einstein obtained the Nobel Prize for Physics in 1921, but for his explanation of the photoelectric effect, not for his brilliant theory of relativity!
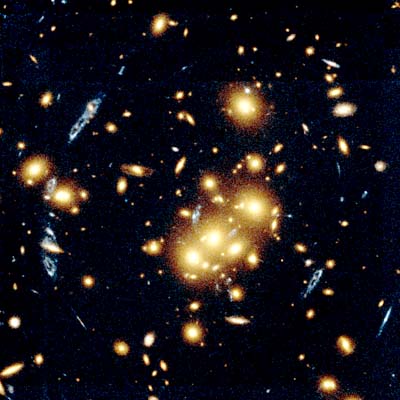
M. Luminet,
Juste pour lire votre article cela va me prendre une journée, je puis dire la plus formidable de toutes. Si plein d’informations pertinentes, pointilleuses et en plus, historiques. Que dire des vidéos… Une mine d’or.
J’ai bien hâte de retrouver la vue de l’oeil droit mal en point, car je dois faire un effort. C’est un plaisir de vous avoir sur facebook et de bénéficier de votre savoir que vous nous offrez gratuitement. Avec vous on ne s’ennuie pas et vous êtes facile à comprendre. On vous lirait et écouterait des heures et des heures.
À la prochaine!
I am fascinated with the total solar eclipse and would like to study more about it and college as well as come up with my own equation for the positioning of the Sun Moon and Earth and the total solar eclipse. Not only that but also how it affects the fabric of space-time and allows Earth to move through space time continuum dust eventually causing us to travel through time backwards not forwards. This is a very brilliant idea and probably closely closely accurate if you can find Newton Tesla’s and Einstein’s theory and eventually you see. The theory that a time machine is possible and the real world but only through heavenly bodies