Sequel of the previous post 40 Years of Black Hole Imaging (2) : Colors and Movies 1989-1993
Generalizations to Kerr Black Holes
Unfortunately Marck’s simulations of black hole accretion disks remained mostly ignored from the professional community, due to the fact that they were not published in peer-reviewed journals and, after their author prematurely died in May 2000, nobody could find the trace of his computer program…
Then, unaware of Marck’s results, several researchers of the 1990’s were involved in the program of calculating black hole gravitational lensing effects in various situations. Stuckey (1993) studied photon trajectories which circle a static black hole one or two times and terminate at their emission points (« boomerang photons »), producing a sequence of ring-shaped mirror images. Nemiroff (1993) described the visual distortion effects to an observer traveling around and descending to the surface of a neutron star and a black hole, discussing multiple imaging, red- and blue-shifting, the photon sphere and multiple Einstein rings. He displayed computer-generated illustrations highlighting the distortion effects on a background stellar field but no accretion disk, and made a short movie now available on the internet (Nemiroff 2018), two snapshots of which are shown in figure 1.
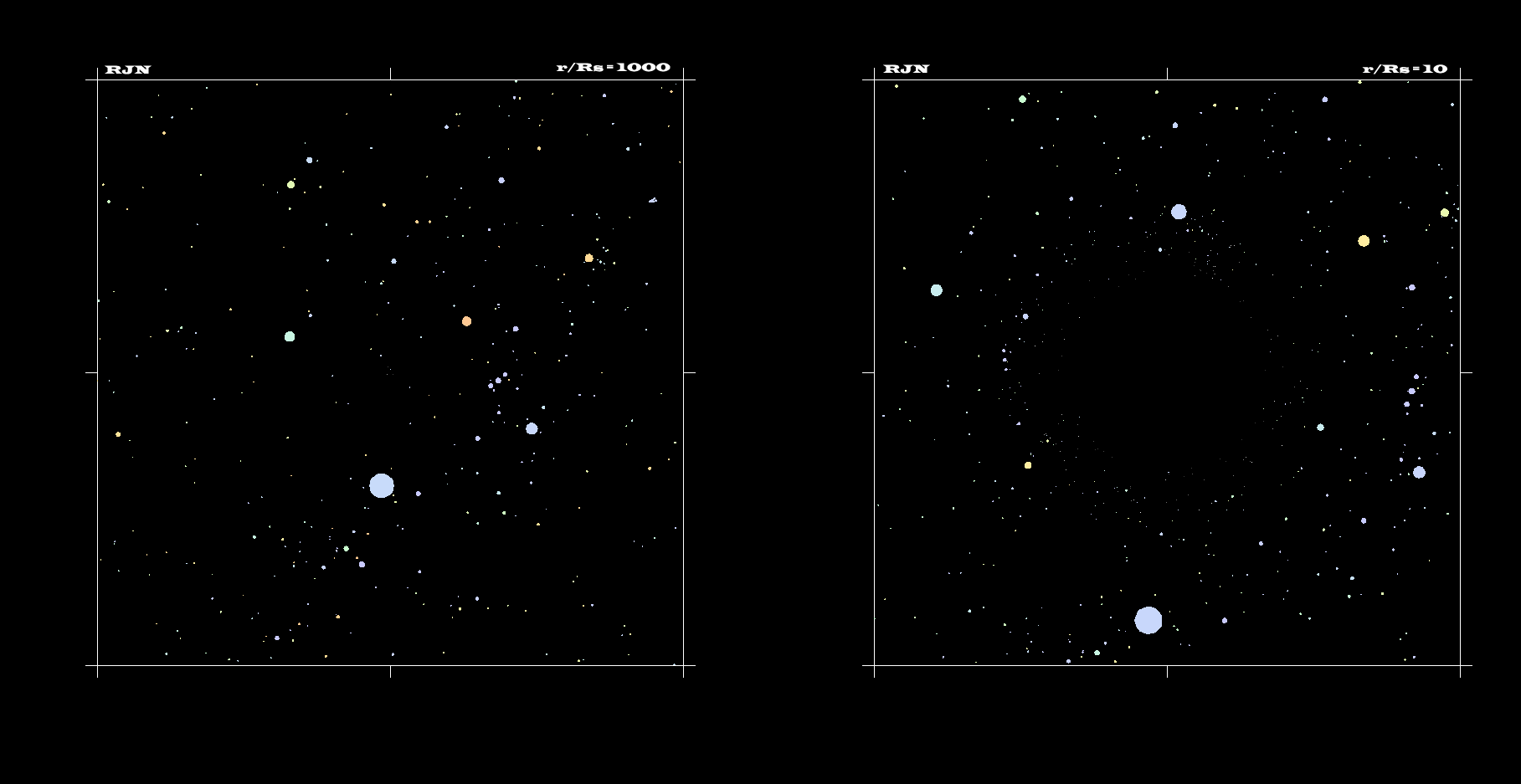
The first simulations of the shape of accretion disks around Kerr black holes were performed by Viergutz (1993). He treated slightly thick disks and produced colored contours, including the disk’s secondary image which wraps under the black hole (figure 2). The result is a colored generalization of the picture by Cunningham and Bardeen (1973) shown in 40 Years of Black Hole Imaging (1).
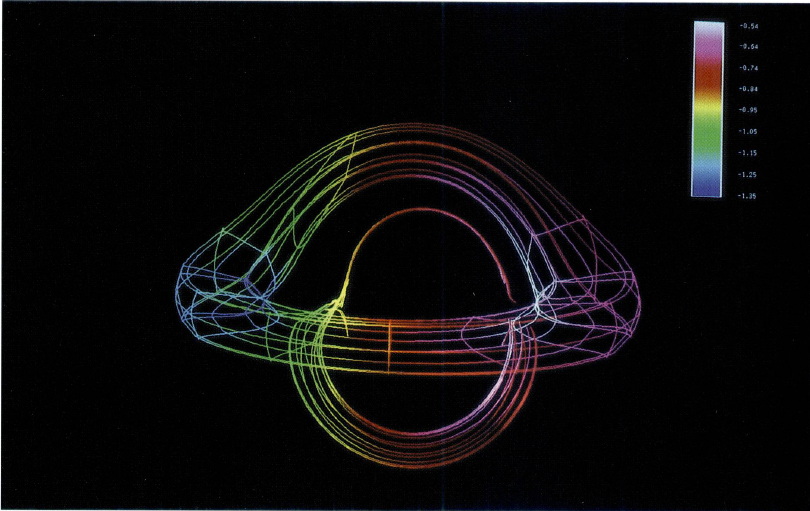
More elaborate views of a geometrically thin and optically thick accretion disk around a Kerr black hole were obtained by Fanton et al. (1997). They developed a new program of ray tracing in Kerr metric, and added false colors to encode the degree of spectral shift and temperature maps (figure 3). Zhang et al. (2002) used the same code to produce black-and-white images of standard thin accretion disks around black holes with different spins, viewing angles and energy bands (figure 4).
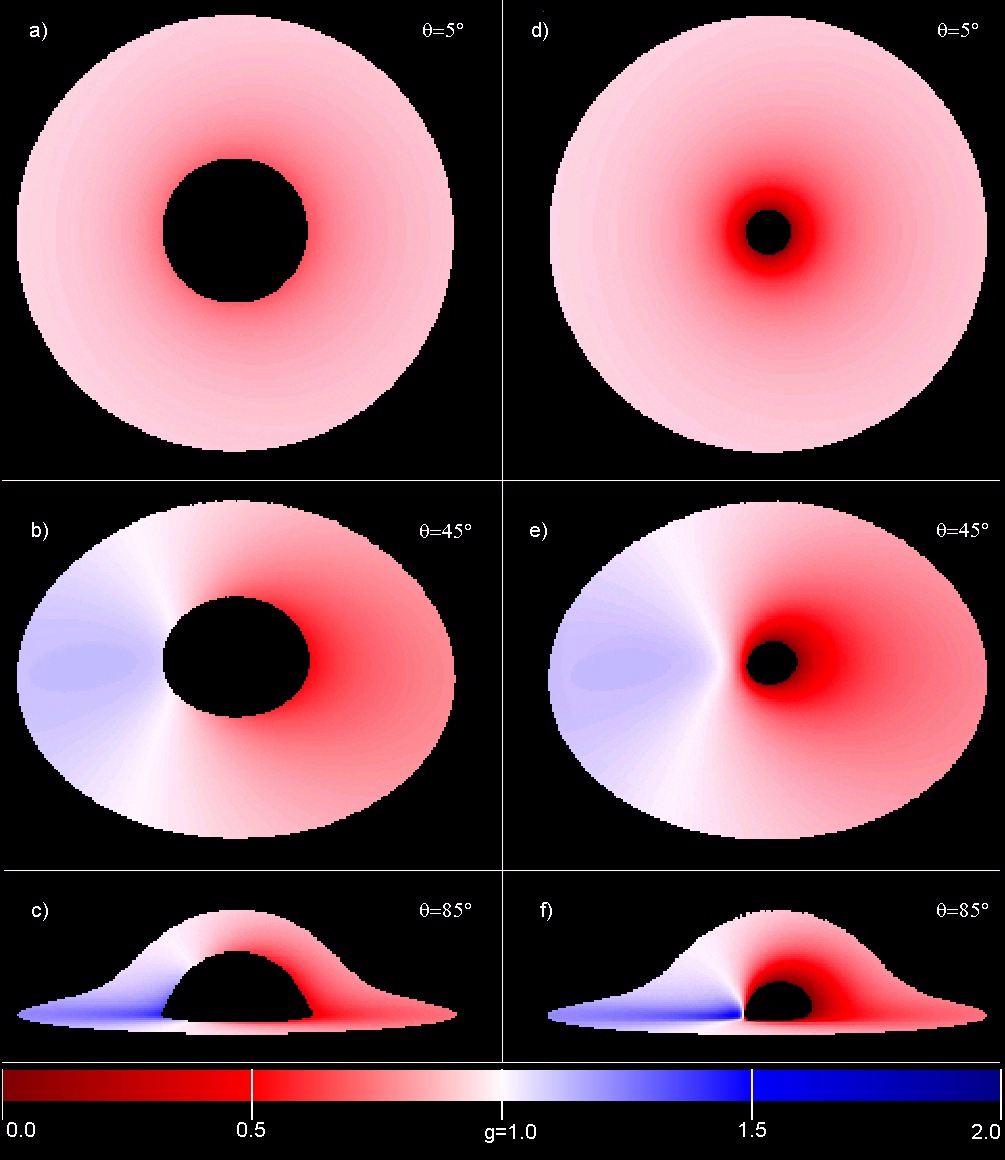
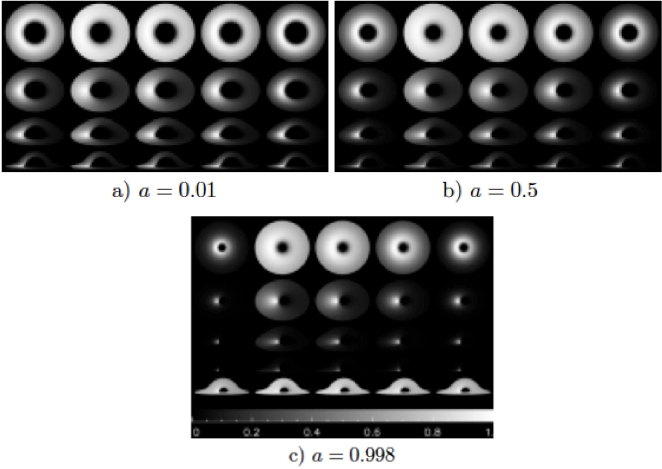
Ben Bromley et al. (1997) calculated integrated line profiles from a geometrically thin disk about a Schwarzschild and an extreme Kerr black hole, in order to get an observational signature of the frame-dragging effect (Figure 5).
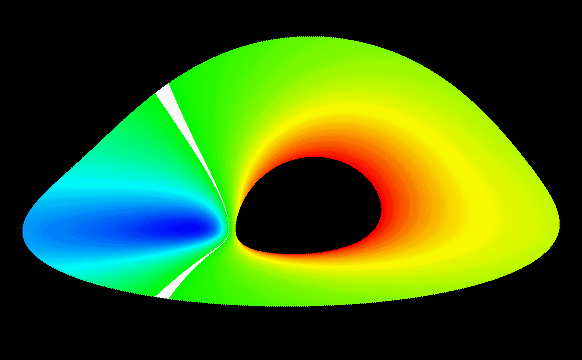
In 1998 Andrew Hamilton started to develop for a student project at the University of Colorado a “Black Hole Flight Simulator”, with film clips that have been shown at planetariums, also available on the Internet. The first depictions were very schematic, but the website was constantly implemented. It now offers journeys into a Schwarzschild or a Reissner-Nordström (i.e. electrically charged) black hole with effects of gravitational lensing on a stellar background field, as well as animated visualizations of magneto-hydrodynamic simulations of a disk and jet around a non-rotating black hole (Hamilton 2018).
Journey into and through an electrically charged (non realistic) Reissner-Nordström black hole, from Andrew Hamilton, 2010
From Idea to Reality
A turning point in the history of black hole imaging came when the possibility of viewing in practice the shadow of SgrA* with VLBI radio astronomy techniques was first discussed (Falcke et al. 2000, Doeleman et al. 2001). Heino Falcke, Fulvio Melia and Eric Agol (who curiously did not quote my 1979 article) developed a general relativistic ray-tracing code that allowed them to simulate observed images of Sgr A* for various combinations of black hole spin, inclination angle, and morphology of the emission region directly surrounding the black hole (figure 6).
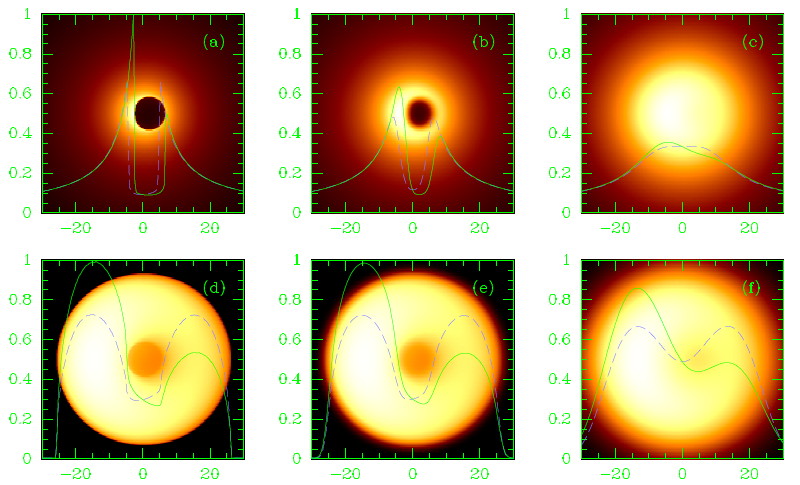
In 2001, Ben Bromley, Fulvio Melia and Siming Liu provided maps of the polarized emission of a Keplerian disk to illustrate how the images of polarized intensity from the vicinity of SgrA* would appear in future VLBI observations (Figure 7).
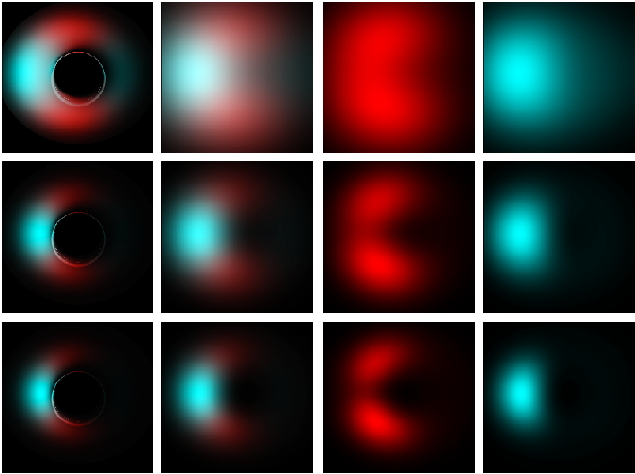
Indeed, in parallel with but rather independently from the theoretical simulations reviewed here, the work to image SgrA* by VLBI experiments had begun also back in the 1970’s, after the discovery of the compact radio source Sgr A* at the center of the Milky Way and its identification as the likely emission of gas falling onto a supermassive black hole (Balick and Brown 1974). And as soon as it was realized that the shadow of SgrA* could really be photographed in the forthcoming years, the program of imaging black holes with or without accretion disks and/or stellar background field developed at a much accelerated rate. Several dozens of papers with more or less elaborate visualizations bloomed out, so many that I’ll stop my illustrated history of black hole imaging at this turning point.
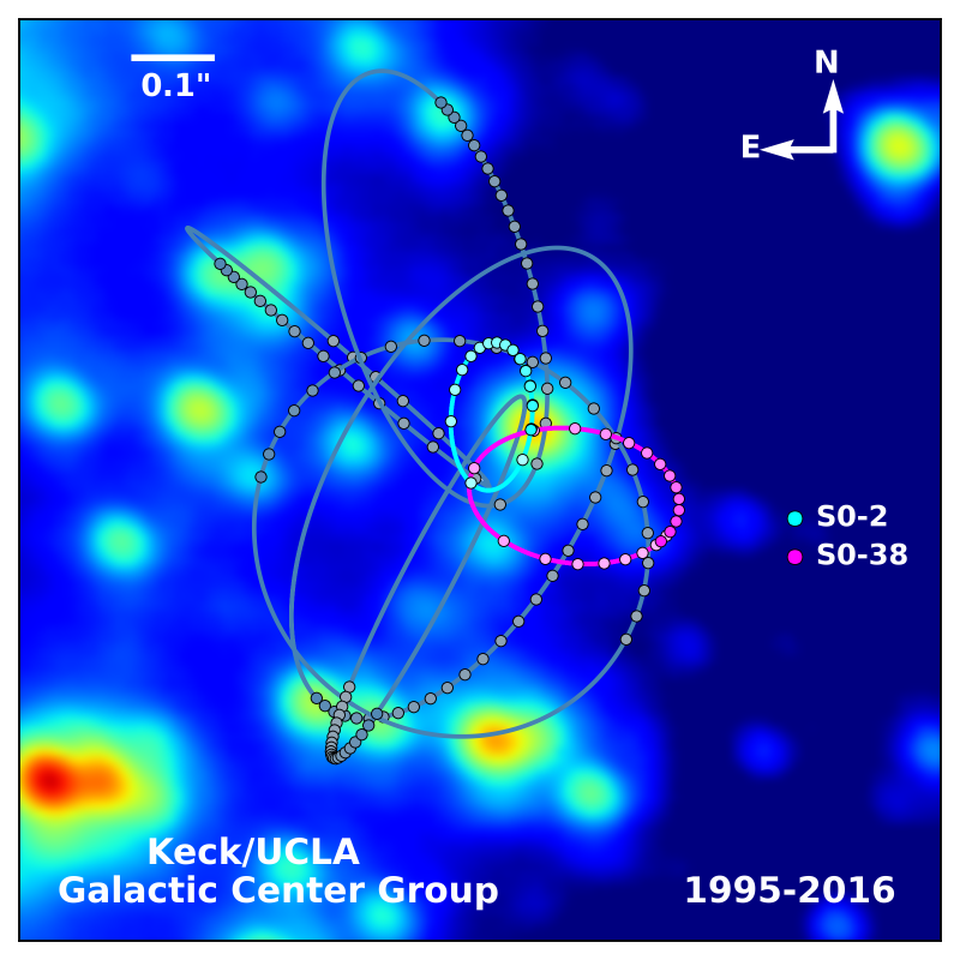
On the observational side, successive radio imaging observations progressively reduced the size of emission region if SgrA*. A breakthrough was to extend VLBI to 1mm wavelength, where the scattering effects are greatly reduced and angular resolution is matched to the shadow of the galactic black hole. Then the collective effort was named the “Event Horizon Telescope” as the natural convergence of many historical and parallel works done by several independent teams in the world (Doeleman et al. 2009). The later measurement of the size of the 6 billion solar mass black hole in M87 gave a second source suitable for shadow imaging (Doeleman et al. 2012).
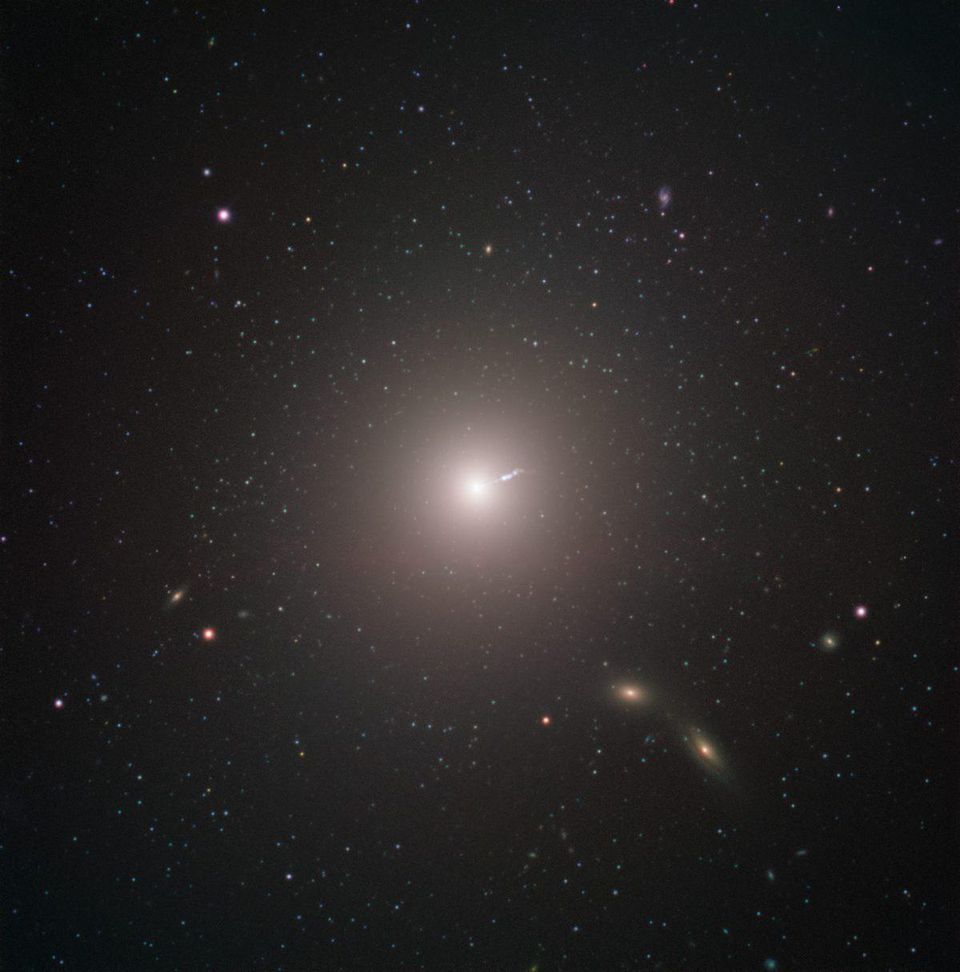
Now the Event Horizon Telescope Consortium involves 20 universities, observatories, research institutions, government agencies and more than a hundred scientists who hope to make black hole imaging a reality as soon as 2019. The first telescopic image of M87* was delivered on April 10th, 2019.
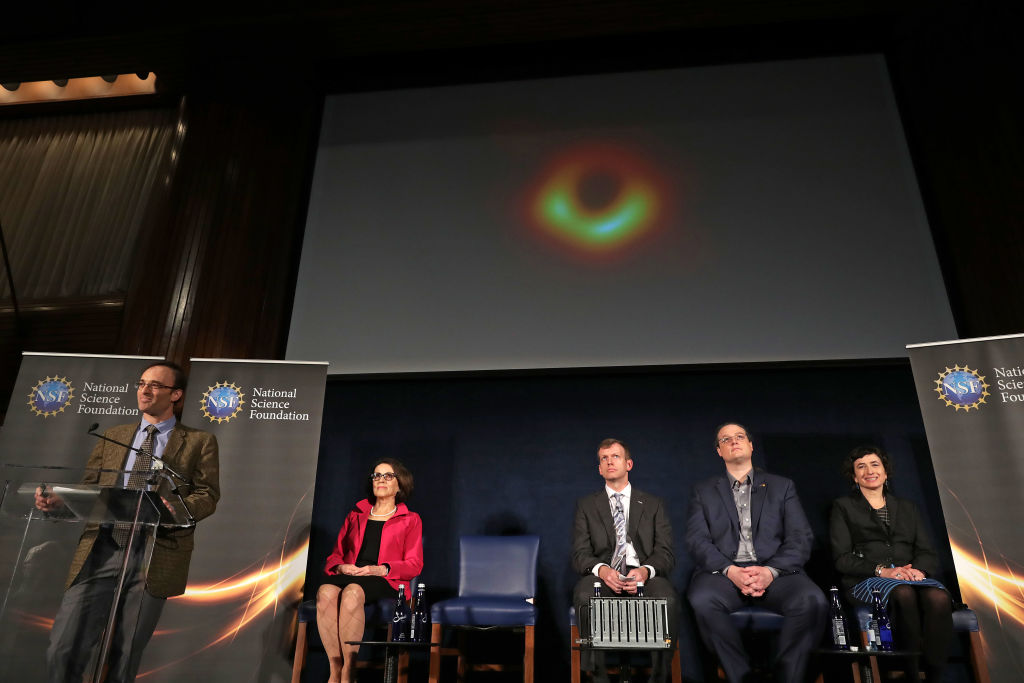
The path from idea to reality can take very a long time. Imaging black holes, first with computers, now with telescopes, is a fantastic adventure. Forty years ago I couldn’t hope that a real image would be reachable in my lifetime and that, thanks to contributions by so many dedicated colleagues, my dream would become true.
In May 2019 I was invited to give the keynote talk at the 3rd Black Hole Initiative Conference at Harvard University and I could warmly congratulate the EHT team. The young commputer scientist Katie Bouman led the development of one of the various algorithms for imaging black holes. We were glad to meet each other, the young and the old !
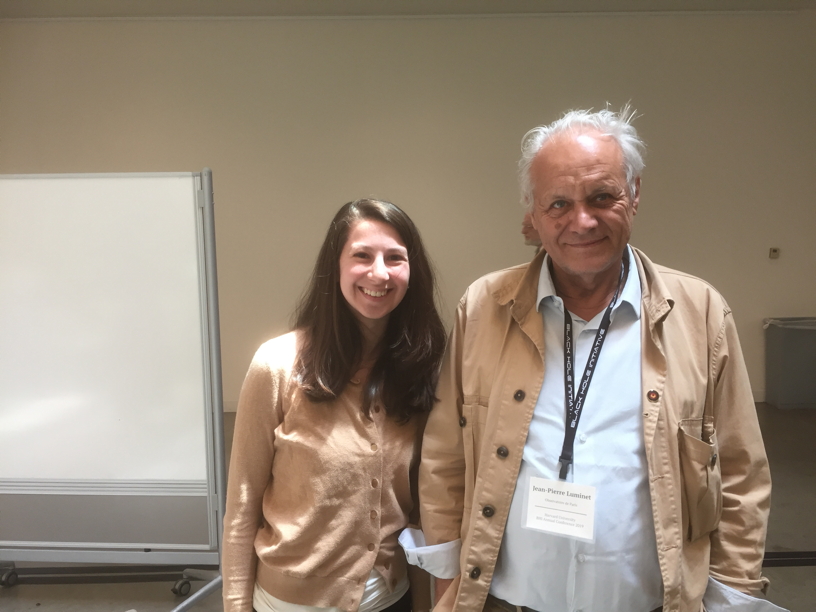
Here is the video of my talk :
Technical References for the 3 posts
Abramowicz, M., Jaroszynski, M., Sikora, M. : Relativistic accreting disks, Astron. Astrophys. 63, 221 (1978).
Balick, B., Brown, R.L. : Intense sub-arcsecond structure in the galactic center, Astrophys. J. 194, 265-270 (1974).
Bardeen, J. M. 1973, Timelike and null geodesics in the Kerr metric, in Black Holes (Les Astres Occlus), ed. C. Dewitt & B. S. Dewitt, New York: Gordon and Breach, pp.215–239.
Bromley, B., Chen, K., Miller,W. : Line Emission from an Accretion Disk around a Rotating Black Hole: Toward a Measurement of Frame Dragging, Astrophys.J. 475, 57 (1997).
Bromley, B., Melia, F., Liu, S. : Polarimetric Imaging of the Massive Black Hole at the Galactic Center, Astrophys.J. 555, L83-86 (2001).
Carter B. : Axisymmetric Black Hole Has Only Two Degrees of Freedom, Physical Review Letters 26, 331 (1971).
Carter B. , Luminet, J.-P. : Les Trous Noirs, Maelströms cosmiques, La Recherche 94, 944 (1978).
Carter B. , Luminet, J.-P. : Pancake Detonation of Stars by Black Holes in Galactic Nuclei, Nature 296, 211 (1982).
Chatzopoulos, S., Fritz, T. K., Gerhard, Gillessen, O., S. , Wegg, C. , Genzel, R. , Pfuhl, O. : The old nuclear star cluster in the Milky Way: dynamics, mass, statistical parallax, and black hole mass, MNRAS, 447, 948 (2015)
Cunningham, C. T. : The effects of redshifts and focusing on the spectrum of an accretion disk around a Kerr black hole, Astrophys. J., 202, 788 (1975)
Cunningham, C.T., Bardeen J.M. : The optical appearance of a star orbiting an extreme Kerr black hole, Astrophys. J.173 L137-142 (1972).
Cunningham, C.T., Bardeen J.M. : The optical appearance of a star orbiting an extreme Kerr black hole, 1973, Astrophys. J., 183, 237
Davelaar, J., Bronzwaer, T., Kok, D., Younsi, Z., Moscibrodzka, M., Falcke, H.: Observing supermassive black holes in virtual reality, Computational Astrophysics and Cosmology 5,1 (2018). https://doi.org/10.1186/s40668-018-0023-7
Delesalle, L. , Lachièze-Rey, M. , Luminet, J.-P. : Infiniment Courbe, TV documentary, 52 mn, France: CNRS/Arte, 1994.
Doeleman, S.S., et al. : Structure of Sagittarius A* at 86 GHz using VLBI closure quantities, Astron. J., 121, 2610-2617 (2001).
Doeleman, S.S., et al. : Event-horizon-scale structure in the supermassive black hole candidate at the Galactic Centre, Nature, 455, 78 (2008).
Doeleman, S.S., et al. : Imaging an Event Horizon : submm-VLBI of Super massive Black Hole, The Astronomy and Astrophysics Decadal Survey, Science White Papers, no. 68 (2009).
Doeleman, S.S., et al. : Jet-Launching Structure Resolved Near the Supermassive Black Hole in M87, Science 338 (6105), 355 (2012).
Doeleman, S.S. : Seeing the unseeable, Nature Astronomy 1, 646 (2017)
Falcke, H. : Imaging black holes : past, present and future, Journal of Physics : Conf. Series 942, 012001 (2017)
Falcke, H., Melia, F., Agol, E. : Viewing the Shadow of the Black Hole at the Galactic Center, Astrophys. J. Lett. 528, L13–L16 (2000).
Fanton C., Calvani M., de Felice F., Cadez A. : Detecting Accretion Disks in Active Galactic Nuclei, Publ. Astron. Soc. Japan 49, 159-169 (1997).
Fukue, J., Yokoyama, T. : Color Photographs of an Accretion Disk around a Black Hole, Publications of the Astronomical Society of Japan 40, 15–24 (1988).
Goddi, C., Falcke, H., et al.: BlackHoleCam: fundamental physics of the galactic center. Int. J. Mod. Phys. D 26, 1730001-239 (2017).
Hamilton, A.: Falling into a black hole. http://jila.colorado.edu/~ajsh/insidebh/intro.html (1998-2018). Accessed 2019-02-26.
Hills, J.G. : Possible power source of Seyfert galaxies and QSOs, Nature 254, 295 (1975).
James, O., von Tunzelmann, E., Franklin, P., Thorne, K.S.: Gravitational lensing by spinning black holes in astrophysics, and in the movie Interstellar. Class. Quantum Gravity 32(6), 065001 (2015).
Johnson, M. D. , Bouman, K. L., Blackburn, L. L. , Chael, A. A., Rosen, J. , Shiokawa, H. , Roelofs, F. , Akiyama, K. , Fish, V. L. , Doeleman S. S. : Dynamical Imaging with Interferometry, Astrophys. J., 850(2), 172 (2017).
Kerr, R.P. : Gravitational Field of a Spinning Mass as an Example of Algebraically Special Metrics, Physical Review Letters 11, 237 (1963).
Kormendy, J., Ho, L. : Supermassive Black Holes in Inactive Galaxies, Encyclopedia of Astronomy and Astrophysics, ed. P.Murdin, article id. 2635. Bristol : Institute of Physics Publishing (2001).
Luminet J.-P.: Seeing Black Holes : from the Computer to the Telescope, Universe 4(8), 86 (2018) [arXiv :1804.03909].
Luminet, J.-P. : Black Holes, Cambridge University Press, 1992.
Luminet, J.-P. : Interstellar Science, International Review of Science vol.1 n°2 (march 2015) [arXiv : 1503.08305].
Luminet, J.-P.: Image of a Spherical Black Hole with Thin Accretion Disk, Astron.Astrophys. 75, 228 (1979).
Marck J.-A., Luminet, J.-P. : Plongeon dans un trou noir, Pour la Science Hors-Série Les trous noirs (July 1997) 50–56.
Marck, J.-A. : Color animation of a black hole with accretion disk, https://www.youtube.com/watch?v=5Oqop50ltrM (1991) (put online in 2011 by J.-P. Luminet).
Marck, J.-A. : Colored images of a black hole accretion disk for various angles of view, unpublished (1989).
Marck, J.-A. : Flight into a Black Hole, videocassette 11 mn, Meudon : CNRS Images (1994).
Marck, J.-A. : Short-Cut Method of Solution of Geodesic Equations for Schwarzschild Black Hole, Classical and Quantum Gravity 13(3) 393–402 (1996).
Marrone, D. P., Moran, J. M., Zhao, J.-H., Rao, R. : An Unambiguous Detection of Faraday Rotation in Sagittarius A*, Astrophys. J. Lett. 654, L57 (2007).
Nemiroff, R. : Visual distortions near a neutron star and black hole, Am. J. Phys. 61, 619 (1993) [astro-ph/9312003].
Nemiroff, R. Trip to a Black Hole https://www.youtube.com/watch?v=ehoOkyHtBXw (2018)
Nerval, G. de : Le Christ aux Oliviers, in Les Chimères, Paris, 1854. Free translation by J.-P. Luminet.
Ohanian, H. C. : The Black Hole as a Gravitational ‘Lens’, Am. J. Phys. 55, 428-432 (1987).
Page, D.N., Thorne, K.S. : Disk Accretion onto a Black Hole. I. Time-Averaged Structure of Accretion Disk, Astrophys. J. 191, 499-506 (1974).
Palmer L., Pryce M. & Unruh W. : Simulation of starlight lensed by a camera orbiting a Schwarzschild black hole, unpublished (1978).
Pounds, K. A. et al. : An ultra-fast inflow in the luminous Seyfert PG1211+143, Monthly Notices of the Royal Astronomical Society 481(2), 1832-1838 (2018).
Pringle, J. E., Rees, M. J. : Accretion Disc Models dor Compact X-ray Sources, Astron. Astrophys. 21 , 1 (1972).
Riazuelo, A. : Seeing relativity I. Ray tracing in a Schwarzschild metric to explore the maximal analytic extension of the metric and making a proper rendering of the stars, (2018) [ArXiv :1511.06025]
Rogers, A. : The Warped Astrophysics of Interstellar, https://www.wired.com/2014/10/astrophysics-interstellar-black-hole/.
Sargent, W. L., Young, P. J., Lynds, C. R., et al. : Dynamical evidence for a central mass concentration in the galaxy M87, Astrophys. J. 221, 731–744 (1978).
Schastok, J. , Soffel, M. , Ruder, H., Schneider, M. : Stellar Sky as Seen From the Vicinity of a Black Hole, Am. J. Phys., 55, 336-341 (1987).
Shaikh, R. : Shadows of rotating wormholes, Phys. Rev. D 98, 024044 (2018) [arXiv :1803.11422]
Shakura, N.I., Sunyaev, R. A. : Black holes in binary systems. Observational appearance. Astro. Astrophys. 24, 337-355 (1973)
Stuckey, W. M. : The Schwarzschild Black Hole as a Gravitational Mirror, Am. J. Phys. 61(5), 448-456 (1993).
Thorne K.: The Science of Interstellar, Norton & Company (november 2014).
Thorne, K, private communication, 24/10/2014
Viergutz S U. : Image Generation in Kerr Geometry. I. Analytical Investigations on the Stationary Emitter-Observer Problem, Astron. Astrophys. 272, 355–77 (1993) ; Viergutz, S.U. : Radiation from arbitrarily shaped objects in the vicinity of Kerr Black Holes, Astrophys. Space Sci. 205, 155 (1993).
Greetings, Are there visualization in other than the optical spectrum? THX Paul